(click here to download a pdf of this bulletin or a one-page synopsis)
In this bulletin, we focus on the implications of climate change for the regeneration of trees and stands, including planting and natural regeneration. Although climate change will affect the ecology and management of our forests on many fronts, its effects on regeneration merit particular attention. Reasons for this include the following:
- Regeneration of trees is central to sustainable forest management;
- Young seedlings are considerably more vulnerable to adverse environmental conditions, including natural disturbances than any other phase in a tree’s development;
- Changes in regeneration are a primary mechanism by which forests acclimate to changes in environmental conditions over the long-term;
- Observed changes in seedling occurrence and abundance can provide important clues on the progress of climate change and potential trajectories of future forest development.
In the following sections, we briefly review some of the more important direct and indirect effects of climate change on regeneration. The final section of the bulletin introduces a number of management implications for the regeneration of forest stands. In the next CSLN bulletin, we shift focus to larger spatial scales, using changes in tree seedling distributions to examine evidence of tree species range shifts.
1. Direct effects of climate change on regeneration
Climate directly influences regeneration through the action of temperature and moisture availability on the biological processes governing each stage of the reproductive cycle, from flowering to seedling growth. By shifting the ‘normal’ range and variability of weather conditions, altering the timing and length of the growing season, climate change will affect (and is already affecting) the ambient environmental conditions in which flowers, cones, and seedlings develop. Additionally, increased frequency of extreme weather events (USGCRP, 2018), such as high temperature extremes and intense rainfall, are expected to cause pulses of mortality among established seedlings, and damage flowers, fruit, and cones before seed development is complete.
Sections below focus on the potential effects of changes in temperature and soil moisture on the multi-stage process that is tree regeneration. Increased atmospheric carbon dioxide concentrations will also affect regeneration by stimulating growth and increasing water use efficiency, but the aggregate long-term effect on plant physiology and physiological processes remains highly uncertain (e.g., McDowell et al., 2020), so is not considered here.
1.1. The regeneration process
The most readily observed effects of climate change on regeneration are changes in seedling growth and survival, but in naturally regenerated stands the seedling phase is the final step in a much longer, climate-dependent process. For species that use sexual reproduction, flowering, cone/seed/fruit development, seed germination, and seedling establishment must all occur before seedling growth and survival become relevant. Climate influences each of these stages of the regeneration process (Ashton and Kelty, 2018). Equally significant, environmental conditions that are optimal for one stage in the regeneration process may not be optimal for other stages in the process (e.g. Savage et al., 1996). This fact has two important implications:
(i) Changes in climate at a given location may improve conditions for some stages of the regeneration process while simultaneously impairing conditions for other stages in the process.
(ii) Changes in climate need only be detrimental to one stage in the regeneration process to cause regeneration failure.
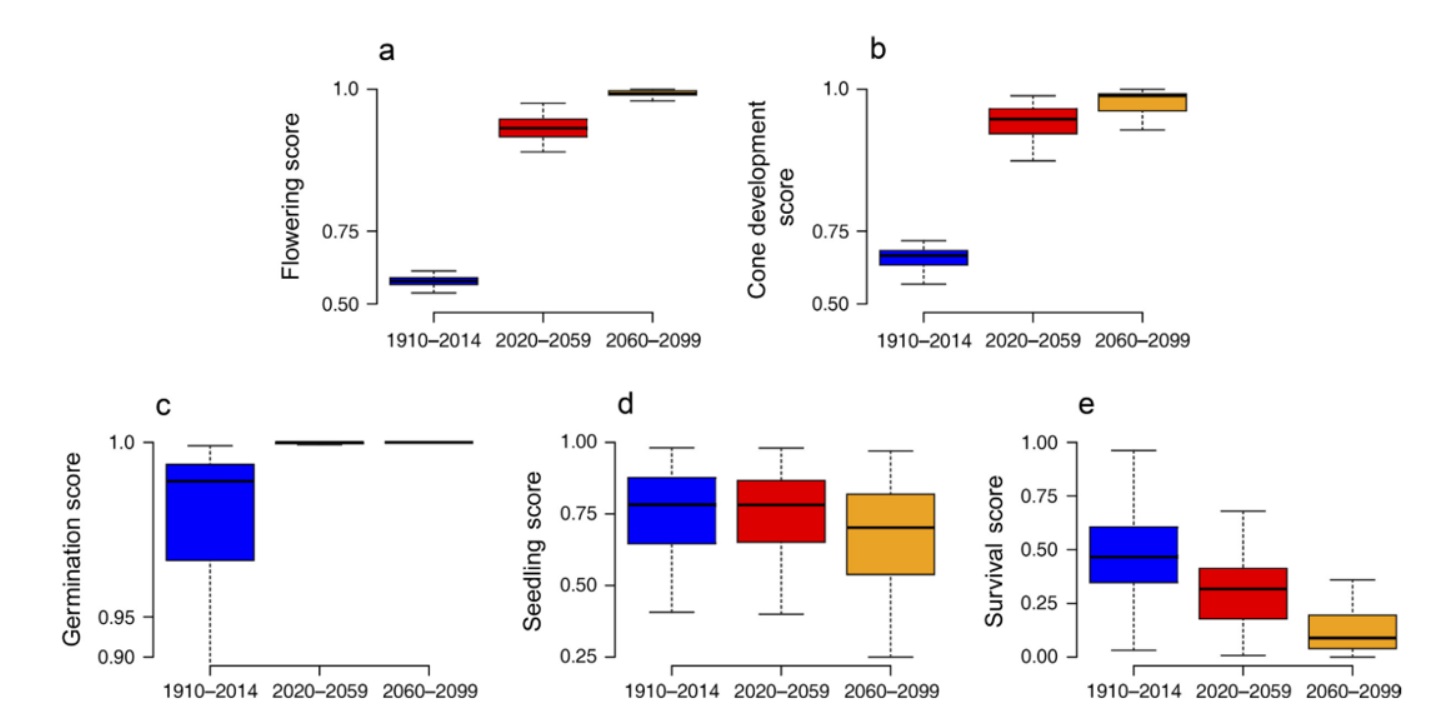
Following these conclusions, it is possible that climate change will have contrasting effects on different stages of the regeneration process for any given species and location. For instance, using ponderosa pine (Pinus ponderosa) physiological tolerances and a climate-water balance model, Petrie et al. (2017) conclude that projected changes in climate at their study sites in western North America may benefit the early stages of the regeneration process, but hinder the later stages (Figure 1). On aggregate, modeled improvements in flowering, cone development, and germination potential outweighed worsening conditions for seedling survival over the period from 2020 – 2059.
By the end of the 21st Century, simulations projected the reverse situation, with extremely high seedling mortality rates offsetting any benefits to seed initiation. It should be noted that this simulation does not include the effects of fire or other disturbances, which are important controls on regeneration in many western dry forests. In a study from eastern North America, Ibañez et al. (2017) found that seed production for red maple (Acer rubrum) and sugar maple (Acer saccharum) was greatest in warmer years, but that establishment and survival rates were highest in cooler years. Models suggested that near-term climate warming may enhance seed production in these species, but depress establishment and survival rates.
These findings demonstrate the need to consider each stage in the reproductive process when evaluating the potential effects of climate change for regeneration. Further, for broadly distributed species, the optimal climate for each stage of the regeneration process may vary across the species range (Boucher et al., 2020; Ibanez et al., 2017), suggesting that the impact of climate change on the regeneration of individual species may also vary according to the location of a site within a species range (Feddema et al., 2013; Bell et al., 2014). While managing for such variability may appear daunting, these facts reinforce the value of a sound understanding of site conditions and tree species biology.
1.2. Changes in temperature
Temperature influences the rate of physiological and environmental processes that directly or indirectly affect reproduction and seedling growth (e.g., photosynthesis, cell division, nutrient transport). As described in Section 1.3, temperature also affects soil moisture levels, so has an indirect impact on plant water status, even in the absence of changes in precipitation regime.
Climate change projections consistently indicate that temperatures will rise across most of North America during the 21st Century, and in a general sense, higher temperatures should have a positive effect on the early phases of the reproductive process (e.g., Kim and Han, 2018; Boucher et al., 2020; Liu and El-Kassaby, 2020). In the absence of other resource constraints, warmer spring temperatures can reduce the time required to complete processes such as flowering and germination. Warmer spring temperatures may also allow these reproductive processes to commence earlier in the year (Classen et al., 2010; Prevéy et al., 2018). Similarly, at higher elevation tree lines, warming that causes earlier snowmelt may improve regeneration potential by extending the growing season (Kroiss and HilleRisLambers, 2014), although it should also be noted that soil moisture limitations (Smithers and North, 2020) and biotic interactions (Lett and Dorrepaal, 2018) may have countervailing effects.
Temperatures during the years preceding germination are also relevant to the regeneration process. Three or more calendar years may pass between flowering and the end of seed maturation in some North American tree species, and cumulative growing degree days during this period may be an important determinant of seed development (Petrie et al, 2017). Production of seed is a resource-intensive process, and warmer conditions during the years preceding flowering and seed production may increase stored carbohydrate reserves for allocation to the reproduction, assuming adequate moisture availability. This is important, as reproduction is typically low in the resource allocation hierarchy. However, the dependency on prior-year temperatures is complex, and some species require a combination of cooler and warmer periods during the years preceding masting (Nussbaumer et al., 2018).
In the absence of other resource constraints, the later stages of the regeneration process – seedling establishment and growth – may also benefit from moderate warming. Higher rates of seedling establishment have been observed under warming treatments in experimental settings in temperate forests (e.g., Fisichelli et al., 2014; Figure 2). Again, assuming moisture requirements have been met, a modest increase in temperature may promote seedling growth, for example, by increasing photosynthetic rates, and extending the growing season (e.g., Peltier and Ibañez, 2015). Rapid early growth could increase survival by reducing exposure to the harsh environmental conditions found at the soil surface (Grossnickle, 2012; Ashton and Kelty, 2018), and may provide seedlings with a competitive advantage over surrounding vegetation (e.g., Carón et al., 2015). This is not always the case, however; in some experimental settings, warmer conditions may increase growth among surviving seedlings, but are also associated with lower survival rates (Fisichelli et al., 2014). Importantly, increased seedling growth in a warmer climate is predicated on sufficient moisture availability. This will be an increasingly important caveat as climate change progresses, and conditions become not only warmer but also more arid (Davis et al., 2019; Rodman et al., 2019).
The benefits of climate warming for regeneration diminish at high temperatures, and winter warming may also be problematic. Rate-limiting reproductive processes often exhibit pronounced temperature optima, above which further increases in temperature are detrimental (Boucher et al., 2020). Germination is the best example of this, with many studies concluding that germination times increase above species-specific optimum temperatures (e.g., Kim and Han, 2018). Extremely high temperatures may also damage the vulnerable tissues of young seedlings (Kolb and Robberecht, 1996; Ashton and Kelty, 2018) and interact with drought to increase plant stress (Allen et al., 2015; Millar and Stephenson, 2015). As the frequency of high temperature extremes is projected to increase across much of North America over the coming century, temperature-related seedling mortality risks are also likely to increase (Vose et al., 2016). These mortality risks are likely to be most acute in existing arid and semi-arid forests in western North America, where low moisture availability combines with high temperatures (Section 1.3). Warmer winters are another threat to successful regeneration for species that employ winter seed dormancy. On sites with moderate winter climates, further warming may inhibit the cold stratification process required to break seed dormancy, delaying or preventing seed germination (Ashton and Kelty, 2018; Prevéy et al., 2018).
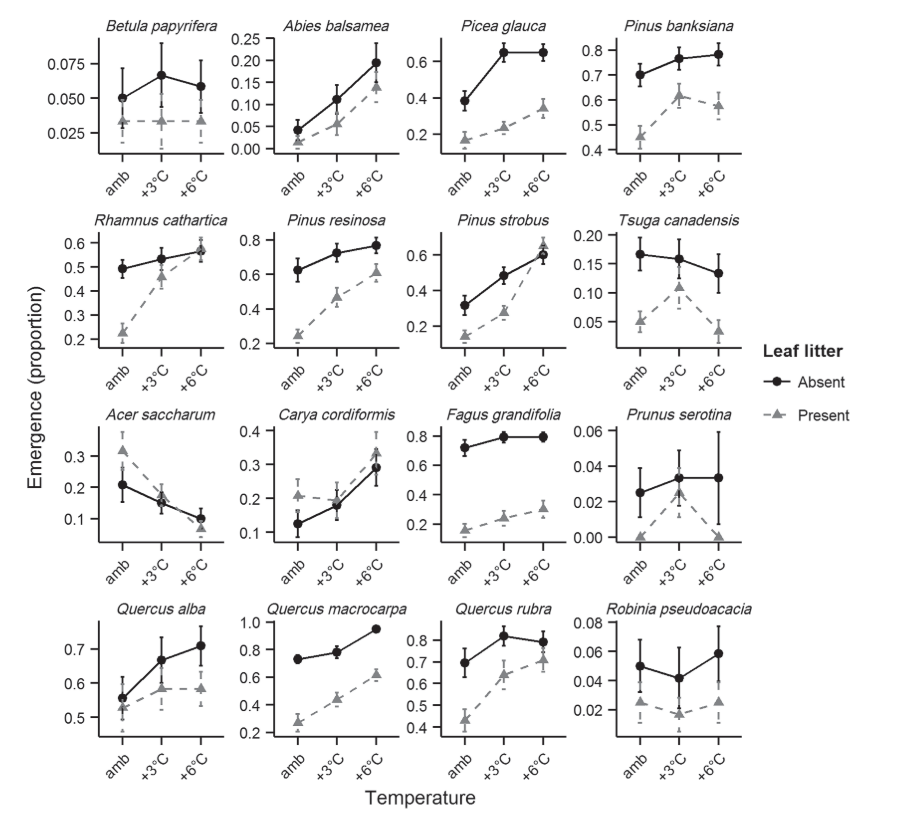
1.3. Changes in moisture availability
All stages of the regeneration process are moisture-sensitive. Soil moisture deficits may cause desiccation and mortality of flowers and seeds, prevent seed germination, hinder seedling establishment, and dramatically reduce growth and survival rates among established seedlings (Rother et al., 2015; Vose et al., 2016; Ashton and Kelty, 2018; Boucher et al., 2020; Kumarathunge et al., 2020).
These effects of inadequate moisture supply are of particular concern given projected reductions in soil moisture across much of North America over the 21st Century. Annual or seasonal soil moisture shortages are already a constraint to growth and driver of extensive overstory mortality in many temperate and boreal forests (Breshears et al., 2005; Allen et al., 2010; Bradford and Bell, 2016). In some regions, further decline in growing season soil moisture is likely to occur as a result of reduced precipitation, earlier snowmelt or more infrequent, but higher intensity rainfall events (Vose et al., 2012; Rother et al., 2015). Reductions in soil moisture during part or all of the year are also likely in areas that experience no change in total precipitation, or even an increase in precipitation. This is a result of climate warming, which drives increased evaporative demand for moisture from the soil surface, in addition to transpiration from plant tissues. Increased moisture loss from the soil reduces moisture availability for vegetation, and this is an acute problem for seedlings due to their small root system, which reduces the soil zone within which water may be obtained (Will et al., 2013; Breshears et al., 2015; Davis et al., 2019). Reductions in soil moisture associated with drought, reduced precipitation, and increased temperature in the absence of changes in precipitation have led to reductions in seedling growth and survival rates during part or all of the year in natural and experimental settings from a range of forest types, including northern temperate and boreal forests (e.g., Erikson et al., 2015; Peltier, D.M. and Ibáñez, I., 2015; Rother et al., 2015) (Figure 2). Seedlings are also particularly vulnerable to the hotter drought phenomenon, in which high temperatures interact with meteorological or hydrological drought to dramatically increase plant stress levels (Allen et al., 2010; Millar and Stephenson, 2015). This can induce mortality directly, or in combination with other stressors, such as disease.
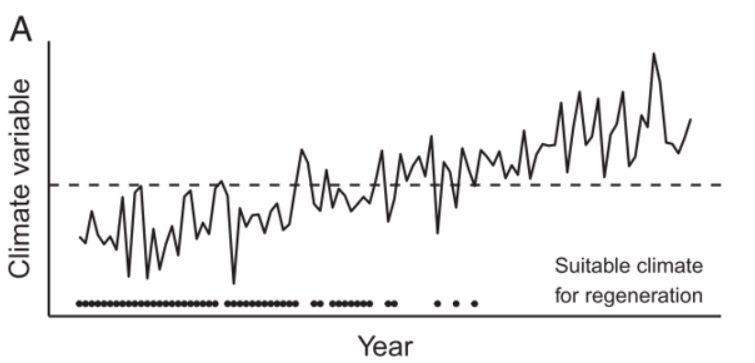
Increased aridity and associated regeneration failures are a particular concern in semi-arid forests of western North America, some of which are currently experiencing one of the most severe long-term drought events of the past 1,200 years (Williams et al., 2013 and 2020). In these relatively moisture-limited environments, regeneration typically occurs in pulses during periods of favorable weather (Petrie et al., 2017; Davis et al., 2019), and increasing aridity during the 21st Century is likely to reduce the frequency of these favorable windows for seedling recruitment (Davis et al., 2019). Over time, it is likely that more and more sites will cross climate thresholds, above which suitable conditions for regeneration will no longer occur (Figure 3). These effects will be compounded by drought-related overstory mortality, which depletes seed sources, and by changes in disturbance regimes (Section 2.2).
Summary
Changes in temperature and moisture availability are among the most important effects of climate change on regeneration. Higher temperatures and associated lengthening of growing seasons may be particularly beneficial to the earlier stages of the regeneration process (flowering through to germination), but realizing the benefits of temperature for regeneration is dependent on an adequate supply of moisture. Soil moisture is critical to all stages of the regeneration process, and reductions in growing season soil moisture are likely in many North American regions over the 21st Century, notably as a consequence of climate warming. The combined effect of changes in temperature and soil moisture on regeneration potential will be highly variable according to location. On some sites, the aggregate effect of reductions in soil moisture associated with warming will be offset by the direct benefits of warmer temperatures (e.g., Fisichelli et al., 2014). On other sites, the reverse will be true, and reductions in soil moisture, potentially combined with periods of extremely hot weather, will outweigh any physiological benefits of warming (e.g., Classen et al., 2010; Kumarathunge et al., 2020). The effects of changes in temperature and soil moisture on regeneration will also vary according to species, even within the same site and of species in the same genus.
Responding to the complex nature of regeneration responses to climate change begins with a sound understanding of site and species. Foundational knowledge for managing climate change impacts on regeneration includes: (i) existing climate and soils on the site; (ii) the biology and silvics of commercially and ecologically important species; (iii) the location and elevation of the site relative to the range of important tree species; and (iv) projected changes in climate.
2. Mediating factors
Climate directly influences regeneration through the action of temperature and moisture availability on the biological processes governing each stage of the reproductive cycle, from flowering to seedling growth. By shifting the ‘normal’ range and variability of weather conditions, altering the timing and length of the growing season, climate change will affect (and is already affecting) the ambient environmental conditions in which flowers, cones, and seedlings develop. Additionally, increased frequency of extreme weather events (USGCRP, 2018), such as high temperature extremes and intense rainfall, are expected to cause pulses of mortality among established seedlings, and damage flowers, fruit, and cones before seed development is complete.
Sections below focus on the potential effects of changes in temperature and soil moisture on the multi-stage process that is tree regeneration. Increased atmospheric carbon dioxide concentrations will also affect regeneration by stimulating growth and increasing water use efficiency, but the aggregate long-term effect on plant physiology and physiological processes remains highly uncertain (e.g., McDowell et al., 2020), so is not considered here.
2.1. Life history traits
Species’ life-history traits are an expression of some dimension of a species’ performance (Violle et al., 2007). In the context of climate change, these life-history traits act like an algorithm, translating changes in the physical environment into realized effects on seedling growth, survival, or competitive status. A large number of functional traits (e.g., germination temperature tolerance) and response traits (e.g., plasticity of leaf morphology) possessed by the parent tree and by seedlings may influence regeneration responses to climate change.
Seedling drought tolerance and shade tolerance are among the most important and well-understood traits that have a bearing on climate change responses. The relevance of shade and drought tolerance to regeneration under climate change is reflected in their use as indices of regeneration potential for management decision-making (Marshall and Falk, 2020). Seedlings of drought tolerant species display superior tolerance to projected reductions in soil moisture availability under climate change (Marshall and Falk, 2020; Minott and Kolb, 2020). Seedlings of species with high shade tolerance typically, although not universally (Monleon and Lintz, 2015), exhibit relatively poor drought tolerance (Niinemets, 2010). Under projected climate change, the benefits of seedling shade tolerance may be most appreciable on sites that are wet to only mildly moisture-limited. On these sites, a shade tolerant seedling’s ability to persist in shaded under-canopy positions yields benefits in avoiding the high temperatures and damaging solar radiation of exposed microsites (Clark et al., 2012; Von Arx, 2013; Hill and Ex, 2020).
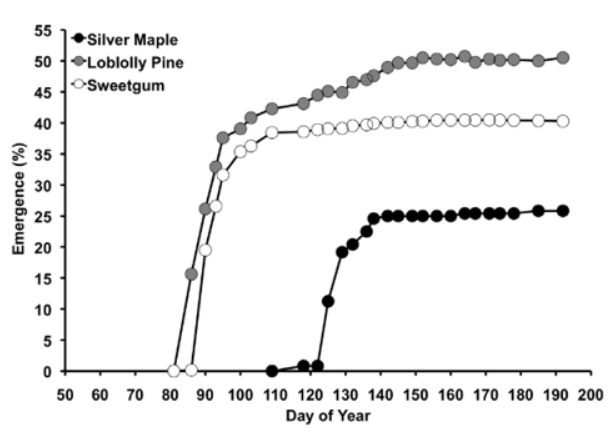
Traits governing seed physiology and germination may also play an important role in determining future forest composition under climate change. Seed dormancy is particularly vulnerable to climate change, and on relatively mild temperate sites, species with this requirement may be at a disadvantage relative to those that do not (Mok et al., 2011; Prevéy et al., 2018). Seed phenology is another potential driver of differential regeneration responses to climate change. For example, the seeds of some species mature in the fall and can begin germination in the spring as soon as temperature and moisture conditions are suitable. The seeds of other species require a maturation period in the early spring before their germination can begin, and on sites that experience summer moisture shortages, this delay may expose seedlings to severe moisture deficits during seedling establishment (Classen et al., 2010) (Figure 4). This example highlights the advantage of a detailed understanding of individual tree species life-history traits, as well as potential changes in site conditions, when planning reforestation activities.
2.2. Natural disturbance
Natural disturbances create conditions for regeneration, but may also be drivers of climate change-induced shifts in seedling composition, regeneration failures, and conversion of forest to non-forest vegetation (Davis et al., 2020; Halofsky et al., 2020). Although a range of disturbances may interact with climate change to influence tree regeneration (Bourgeade et al., 2018; Lucash et al., 2018), the relationship between climate change and fire – notably high-severity fire – has particularly significant implications for tree regeneration, and has become an important research theme over the past five years. Central to this relationship is (i) the role of climate change as a driver of ongoing changes in fire regimes (e.g., Williams et al., 2019), and (ii) the effect of climate change on regeneration conditions following wildfire (e.g., Stevens-Rumann et al., 2018).
Interactions between climate change and fire may shape regeneration outcomes in the following ways (amongst many others) (Stevens-Rumann et al., 2018; Davis et al., 2019; Coop et al., 2020; Halofsky et al., 2020):
- High-severity wildfire may accelerate climate change-induced shifts in forest composition in naturally regenerated stands by creating growing space for species able to regenerate under more arid contemporary conditions than those that existed during origination of the previous stand.
- Increasing fire frequency in naturally regenerated stands may deplete seedbanks and prevent seedlings from attaining seed-producing age before subsequent fires. These changes shift the competitive balance toward species with frequent-fire adaptations, and increase the potential for conversion from forest to non-forest vegetation.
- Increasing high-severity fire patch size (i.e. the contiguous area burned at high-severity) increases the distance to seed source, which imposes a major constraint to natural regeneration.
- Extremely high-severity fires may alter soil surface properties in ways that increase the biological impediments to regeneration of planted or naturally regenerated seedlings following fire.
- Increasing aridity associated with climate change increases the biological impediments to regeneration of natural or planted seedlings following fire.
There is particular concern over regeneration failure where periods of drought and extreme temperature follow high-severity fire. Under these conditions, regeneration of both natural and planted seedlings becomes challenging, mortality of any residual overstory trees further depletes natural seed sources, and additional inputs of dead wood may increase the likelihood of reburns (Rother et al., 2015; Littell et al., 2016; Halofsky et al., 2020).
3. Implications for the regeneration of stands
Our brief review of the implications of climate change for regeneration provides some illustration of the many different pathways by which climate change effects may be felt, and the variability of these effects from species to species and site to site. Below, we synthesize a number of broadly applicable implications for managing the regeneration of trees and stands under the influence of climate change. We do not attempt to review detailed elements of planted seedling cultivation in nurseries, as this has been covered elsewhere (e.g., Grossnickle, 2012; Grossnickle and MacDonald, 2018). Instead, we focus on conditions in the forest. It is essential to note that these generalized recommendations provide a starting point for developing site-specific management options. As is the case with other elements of climate change adaptation, management interventions to maintain or improve regeneration potential should be determined on the basis of individual site-scale vulnerabilities and opportunities, and in the context of evolving scientific understanding.
3.1. The most important implication of all!
The most important implication, and the most generalizable of all, is the importance of local knowledge. This has been noted throughout this review and is worth reiterating. Managing the impact of climate change on regeneration should begin with (i) a detailed understanding of the site, the biology, and silvics of commercially and ecologically important species, and potential changes in climate; and (ii) monitoring ongoing changes in forest structure, composition and function; and (iii) evaluation of the potential impact of change relative to pre-existing management objectives (Swanston et al., 2016).
3.2. Silvicultural systems and harvest planning
Achieving successful regeneration is fundamental to the design of silvicultural systems, and adjusting the silvicultural system is an obvious starting point for building resilience into the regeneration process.
- On harsh sites that are projected to experience increasingly challenging regeneration conditions, the use of planted seedlings instead of natural regeneration should be considered. Planting increases control over regeneration quantity, quality, and spatial arrangement, each of which may be crucial in overcoming climate change stressors. In some circumstances, the financial penalty involved in switching from natural regeneration to planted stock may be offset by increased seedling survival, reduced time required for regeneration, and at the extreme, maintaining the site as forestland.
- Adjusting the amount and spatial arrangement of green tree retention may increase the likelihood of successful regeneration. From a regeneration perspective, decisions on the optimal amount of retention should be made on the basis of existing and potential future conditions over the time to seedling maturation, and species-specific shade, drought, and temperature tolerance. In some cases, changes in retention levels may be counter-intuitive, so decisions-making should consider both existing and emerging knowledge of species-specific responses to climate stressors. For example, on some extremely dry sites, increasing retention levels may – counterintuitively – benefit species with high intolerance of shade (Hill and Ex, 2020; Steed and Goeking, 2020). In management systems that rely on natural regeneration, distance to seed source, potential disturbance risks to these seed sources, and climate change risks to flower, cone, and seed development are all important determinants of regeneration outcomes and should be considered during harvest planning.
- Where tree planting is employed, adjusting the timing of planting, at annual and sub-annual timescales, may alter regeneration outcomes. At annual timescales, incorporating the timing of El Niño Southern Oscillation events into harvest planning provides an opportunity to synchronize planting on dry/wet sites with years in which above/below average precipitation or temperature are more likely (Rother et al., 2015). At sub-annual timescales, understanding and monitoring changes in seasonal temperature and precipitation can help identify optimal months for planting. Allied with this, maintaining close relationships with providers of planting stock can improve responsiveness and flexibility to take advantage of desirable planting periods (Dumroese et al., 2016).
3.3. Microsite conditions
Modifying the seedbed to improve regeneration conditions is central to traditional silviculture, and this knowledge can be used to advantage in the face of climate change.
- Shelter may be the most relevant component of the microsite to coping with increasingly harsh conditions associated with a warming climate. Retaining live trees (whether in uneven-aged systems or harvest reserves in even-aged systems) ameliorate conditions at the soil surface for seedlings, but living vegetation also reduces available soil moisture, which can be particularly problematic during drought periods (Ashton and Kelty, 2018; Minott and Kolb, 2020). Snags, logs, and stumps do not compete for moisture, and can moderate local microclimate at the soil surface. As a result, retaining coarse, woody debris may enhance seedling survival rates during periods of severe environmental conditions (Hill and Ex, 2020). For some timber species, logs and stumps are also preferred regeneration sites, contributing moisture and nutrients (Harmon et al., 1986; Bolton and D’Amato, 2011). For these reasons, the retention of dead structures within the harvest unit may actively contribute to regeneration goals under projected climate warming.
- Adjusting harvest unit configuration provides another means of influencing conditions at the soil surface for natural regeneration and planted seedlings. Changes in harvest unit dimensions and their relationship with site topography will alter the zone of influence of gap-edge trees, and thereby change the regeneration niche (Ashton and Kelty, 2018). Experimentation with such attributes may yield benefits for seedling growth and survival.
3.4. Species mix
Combine new and existing knowledge of site and species to improve regeneration outcomes.
- In planted stands, match reforestation species to current and projected future conditions and enhance diversity. By combining informed estimates of projected climate change impacts on the physical site with knowledge of the silvics of native timber species, it may be possible to adjust the planting mix so as to minimize planted seedling vulnerabilities. This is not a call for assisted migration, the evidence for which remains limited at present. Instead, it is an encouragement to consider the most site-appropriate native commercial timber species or provenances. For example, use the most drought-tolerant of the available species/provenances in plantings on currently moisture-limited south-facing slopes, or on soils with poor water-holding capacity. Increasing species diversity of the planting mix, or using provenances that have broad climatic tolerances are other common recommendations in the face of dynamic and uncertain future conditions. Increasing species diversity at the meso-, stand-, and sub-stand scales may previously have been an inconvenience. However, on some sites, projected changes in climate should prompt a re-evaluation of pre-existing values in light of the potential risks of regeneration failure. Adopting a flexible approach to regeneration that is focused on attainable management objectives, not specific species, may facilitate adaptability to changing conditions.
- In naturally-regenerated mixed-species stands, understand the silvics of existing timber species while recognizing the dynamic nature of competitive interactions. Changes in the relative dominance of species in the regeneration layer can be expected as a result of changes in site conditions that favor one or more species over their competitors. Additionally, there is evidence that novel competitive interactions may emerge as seedlings adapt to changing conditions, and this too may influence subsequent stand structure. Monitoring of regeneration dynamics can identify these interactions to inform future management.
3.5. Regeneration failures
While climate change will undoubtedly increase the probability of successful regeneration for certain species on certain sites, securing adequate levels of regeneration will become increasingly challenging on other sites. On sites in which regeneration is problematic, appropriate institutional responses include:
- Recognizing that climate change brings increasing risks of regeneration failure on many sites, and where appropriate, incorporate this into financial models.
- Increasing the intensity of post-planting monitoring on all sites for which regeneration failure is identified as a potential scenario.
_ _ _ _ _
References
Allen, C.D., Macalady, A.K., Chenchouni, H., Bachelet, D., McDowell, N., Vennetier, M., Kitzberger, T., Rigling, A., Breshears, D.D., Hogg, E.T. and Gonzalez, P., 2010. A global overview of drought and heat-induced tree mortality reveals emerging climate change risks for forests. Forest Ecology and Management, 259(4), pp.660-684.
Ashton, M.S. and Kelty, M.J., 2018. The practice of silviculture: applied forest ecology, 10th Edition. John Wiley & Sons Ltd., Hoboken, New Jersey.
Bell, D.M., Bradford, J.B. and Lauenroth, W.K., 2014. Early indicators of change: divergent climate envelopes between tree life stages imply range shifts in the western United States. Global Ecology and Biogeography, 23(2), pp.168-180.
Bolton, N.W. and D’Amato, A.W., 2011. Regeneration responses to gap size and coarse woody debris within natural disturbance-based silvicultural systems in northeastern Minnesota, USA. Forest Ecology and Management, 262(7), pp.1215-1222.
Boucher, D., Gauthier, S., Thiffault, N., Marchand, W., Girardin, M. and Urli, M., 2019. How climate change might affect tree regeneration following fire at northern latitudes: a review. New Forests, pp.1-29.
Bourgeade, P., Bourioug, M., Macor, S., Alaoui-Sossé, L., Alaoui-Sossé, B. and Aleya, L., 2018. Potential vulnerability of oak forests to climate change-induced flooding: effects of mild oxygen deficiency on Quercus robur and Quercus petraea seedling physiology. Environmental Science and Pollution Research, 25(6), pp.5550-5557.
Bradford, J.B. and Bell, D.M., 2017. A window of opportunity for climate‐change adaptation: easing tree mortality by reducing forest basal area. Frontiers in Ecology and the Environment, 15(1), pp.11-17.
Breshears, D.D., Cobb, N.S., Rich, P.M., Price, K.P., Allen, C.D., Balice, R.G., Romme, W.H., Kastens, J.H., Floyd, M.L., Belnap, J. and Anderson, J.J., 2005. Regional vegetation die-off in response to global-change-type drought. Proceedings of the National Academy of Sciences, 102(42), pp.15144-15148.
Breshears, D.D., Adams, H.D., Eamus, D., McDowell, N., Law, D.J., Will, R.E., Williams, A.P. and Zou, C.B., 2013. The critical amplifying role of increasing atmospheric moisture demand on tree mortality and associated regional die-off. Frontiers in Plant Science, 4, p.266.
Carón, M.M., De Frenne, P., Brunet, J., Chabrerie, O., Cousins, S.A., Decocq, G., Diekmann, M., Graae, B.J., Heinken, T., Kolb, A. and Lenoir, J., 2015. Divergent regeneration responses of two closely related tree species to direct abiotic and indirect biotic effects of climate change. Forest Ecology and Management, 342, pp.21-29.
Chapin III, F.S., Matson, P.A. and Vitousek, P., 2011. Principles of Terrestrial Ecosystem Ecology. Springer Science & Business Media.
Clark, J.S., Bell, D.M., Kwit, M., Stine, A., Vierra, B. and Zhu, K., 2012. Individual-scale inference to anticipate climate-change vulnerability of biodiversity. Philosophical Transactions of the Royal Society B: Biological Sciences, 367(1586), pp.236-246.
Classen, A.T., Norby, R.J., Sides, K.E. and Weltzin, J.F., 2010. Climate change alters seedling emergence and establishment in an old-field ecosystem. PLoS One, 5(10), p.e13476.
Coop, J.D., Parks, S.A., Stevens-Rumann, C.S., Crausbay, S.D., Higuera, P.E., Hurteau, M.D., Tepley, A., Whitman, E., Assal, T., Collins, B.M. and Davis, K.T., 2020. Wildfire-driven forest conversion in western North American landscapes. BioScience, 70(8), pp.659-673.
Davis, K.T., Dobrowski, S.Z., Higuera, P.E., Holden, Z.A., Veblen, T.T., Rother, M.T., Parks, S.A., Sala, A. and Maneta, M.P., 2019. Wildfires and climate change push low-elevation forests across a critical climate threshold for tree regeneration. Proceedings of the National Academy of Sciences, 116(13), pp.6193-6198.
Davis, K.T., Higuera, P.E., Dobrowski, S.Z., Parks, S.A., Abatzoglou, J.T., Rother, M.T. and Veblen, T.T., 2020. Fire-catalyzed vegetation shifts in ponderosa pine and Douglas-fir forests of the western United States. Environmental Research Letters, 15(10), p.1040b8.
Dobrowski, S.Z., Swanson, A.K., Abatzoglou, J.T., Holden, Z.A., Safford, H.D., Schwartz, M.K. and Gavin, D.G., 2015. Forest structure and species traits mediate projected recruitment declines in western US tree species. Global Ecology and Biogeography, 24(8), pp.917-927.
Dumroese, K.R., Landis, T.D., Pinto, J.R., Haase, D.L., Wilkinson, K.W. and Davis, A.S., 2016. Meeting forest restoration challenges: using the target plant concept. Reforesta, 1(1), pp.37-52.
Erickson, A., Nitschke, C., Coops, N., Cumming, S. and Stenhouse, G., 2015. Past-century decline in forest regeneration potential across a latitudinal and elevational gradient in Canada. Ecological Modelling, 313, pp.94-102.
Evans, P., Crofts, A.L. and Brown, C.D., 2020. Biotic filtering of northern temperate tree seedling emergence in beyond‐range field experiments. Ecosphere, 11(5), p.e03108.
Feddema, J.J., Mast, J.N. and Savage, M., 2013. Modeling high-severity fire, drought and climate change impacts on ponderosa pine regeneration. Ecological Modelling, 253, pp.56-69.
Fisichelli, N., Wright, A., Rice, K., Mau, A., Buschena, C. and Reich, P.B., 2014. First‐year seedlings and climate change: species‐specific responses of 15 North American tree species. Oikos, 123(11), pp.1331-1340.
Grossnickle, S.C., 2012. Why seedlings survive: influence of plant attributes. New Forests, 43(5-6), pp.711-738.
Grossnickle, S.C. and MacDonald, J.E., 2018. Why seedlings grow: influence of plant attributes. New Forests, 49(1), pp.1-34.
Halofsky, J.E., Peterson, D.L. and Harvey, B.J., 2020. Changing wildfire, changing forests: the effects of climate change on fire regimes and vegetation in the Pacific Northwest, USA. Fire Ecology, 16(1), p.4.
Harmon, M.E., Franklin, J.F., Swanson, F.J., Sollins, P., Gregory, S.V., Lattin, J.D., Anderson, N.H., Cline, S.P., Aumen, N.G., Sedell, J.R. and Lienkaemper, G.W., 1986. Ecology of coarse woody debris in temperate ecosystems. Advances in Ecological Research, 15, pp.133-302.
Hill, E.M. and Ex, S., 2020. Microsite conditions in a low-elevation Engelmann spruce forest favor ponderosa pine establishment during drought conditions. Forest Ecology and Management, 463, p.118037.
Ibáñez, I., Katz, D.S. and Lee, B.R., 2017. The contrasting effects of short-term climate change on the early recruitment of tree species. Oecologia, 184(3), pp.701-713.
Kim, D.H., and Han, S.H., 2018. Direct effects on seed germination of 17 tree species under elevated temperature and CO2 conditions. Open life Sciences, 13(1), pp.137-148.
Kolb, P.F. and Robberecht, R., 1996. High temperature and drought stress effects on survival of Pinus ponderosa seedlings. Tree Physiology, 16(8), pp.665-672.
Kroiss, S.J. and HilleRisLambers, J., 2015. Recruitment limitation of long‐lived conifers: implications for climate change responses. Ecology, 96(5), pp.1286-1297.
Kumarathunge, D.P., Drake, J.E., Tjoelker, M.G., López, R., Pfautsch, S., Vårhammar, A. and Medlyn, B.E., 2020. The temperature optima for tree seedling photosynthesis and growth depend on water inputs. Global Change Biology, 26(4), pp.2544-2560.
Lett, S. and Dorrepaal, E., 2018. Global drivers of tree seedling establishment at alpine treelines in a changing climate. Functional Ecology, 32(7), pp.1666-1680.
Littell, J.S., Peterson, D.L., Riley, K.L., Liu, Y. and Luce, C.H., 2016. A review of the relationships between drought and forest fire in the United States. Global Change Biology, 22(7), pp.2353-2369.
Liu, Y. and El-Kassaby, Y.A., 2020. Ecological drivers of plant life-history traits: Assessment of seed mass and germination variation using climate cues and nitrogen resources in conifers. Ecological Indicators, 117, p.106517.
Lucash, M.S., Scheller, R.M., Sturtevant, B.R., Gustafson, E.J., Kretchun, A.M. and Foster, J.R., 2018. More than the sum of its parts: how disturbance interactions shape forest dynamics under climate change. Ecosphere, 9(6), p.e02293.
Marshall, L.A. and Falk, D.A., 2020. Demographic trends in community functional tolerance reflect tree responses to climate and altered fire regimes. Ecological Applications, 30(8), p.e02197.
Millar, C.I. and Stephenson, N.L., 2015. Temperate forest health in an era of emerging megadisturbance. Science, 349(6250), pp.823-826.
Minott, J.A. and Kolb, T.E., 2020. Regeneration patterns reveal contraction of ponderosa forests and little upward migration of pinyon-juniper woodlands. Forest Ecology and Management, 458, p.117640.
Mok, H.F., Arndt, S.K. and Nitschke, C.R., 2012. Modelling the potential impact of climate variability and change on species regeneration potential in the temperate forests of South‐Eastern Australia. Global Change Biology, 18(3), pp.1053-1072.
Monleon, V.J. and Lintz, H.E., 2015. Evidence of tree species’ range shifts in a complex landscape. PLoS One, 10(1), p.e0118069.
Niinemets, Ü., 2010. Responses of forest trees to single and multiple environmental stresses from seedlings to mature plants: past stress history, stress interactions, tolerance and acclimation. Forest Ecology and management, 260(10), pp.1623-1639.
Nussbaumer, A., Waldner, P., Apuhtin, V., Aytar, F., Benham, S., Bussotti, F., Eichhorn, J., Eickenscheidt, N., Fabianek, P., Falkenried, L. and Leca, S., 2018. Impact of weather cues and resource dynamics on mast occurrence in the main forest tree species in Europe. Forest Ecology and Management, 429, pp.336-350.
Peltier, D.M. and Ibáñez, I., 2015. Patterns and variability in seedling carbon assimilation: implications for tree recruitment under climate change. Tree Physiology, 35(1), pp.71-85.
Petrie, M.D., Bradford, J.B., Hubbard, R.M., Lauenroth, W.K., Andrews, C.M. and Schlaepfer, D.R., 2017. Climate change may restrict dryland forest regeneration in the 21st century. Ecology, 98(6), pp.1548-1559.
Prevéy, J.S., Harrington, C.A. and Clair, J.B.S., 2018. The timing of flowering in Douglas-fir is determined by cool-season temperatures and genetic variation. Forest Ecology and Management, 409, pp.729-739.
Rodman, K.C., Veblen, T.T., Battaglia, M.A., Chambers, M.E., Fornwalt, P.J., Holden, Z.A., Kolb, T.E., Ouzts, J.R. and Rother, M.T., 2020. A changing climate is snuffing out post‐fire recovery in montane forests. Global Ecology and Biogeography, 29(11), pp.2039-2051.
Rother, M.T., Veblen, T.T. and Furman, L.G., 2015. A field experiment informs expected patterns of conifer regeneration after disturbance under changing climate conditions. Canadian Journal of Forest Research, 45(11), pp.1607-1616.
Savage, M., Brown, P.M. and Feddema, J., 1996. The role of climate in a pine forest regeneration pulse in the southwestern United States. Ecoscience, 3(3), pp.310-318.
Saxe, H. and Kerstiens, G., 2005. Climate change reverses the competitive balance of ash and beech seedlings under simulated forest conditions. Plant Biology, 7(4), pp.375-386.
Smithers, B.V. and North, M.P., 2020. Mechanisms of species range shift: germination and early survival of Great Basin bristlecone pine and limber pine. Plant and Soil, 457(1), pp.167-183.
Steed, J.E. and Goeking, S.A., 2020. Western larch regeneration responds more strongly to site and indirect climate factors than to direct climate factors. Forests, 11(4), p.482.
Stevens‐Rumann, C.S., Kemp, K.B., Higuera, P.E., Harvey, B.J., Rother, M.T., Donato, D.C., Morgan, P. and Veblen, T.T., 2018. Evidence for declining forest resilience to wildfires under climate change. Ecology letters, 21(2), pp.243-252.
Swanston, Christopher W.; Janowiak, Maria K.; Brandt, Leslie A.; Butler, Patricia R.; Handler, Stephen D.; Shannon, P. Danielle; Derby Lewis, Abigail; Hall, Kimberly; Fahey, Robert T.; Scott, Lydia; Kerber, Angela; Miesbauer, Jason W.; Darling, Lindsay; Parker, Linda; St. Pierre, Matt. 2016. Forest Adaptation Resources: climate change tools and approaches for land managers, 2nd ed. Gen. Tech. Rep. NRS-GTR-87-2. Newtown Square, PA: U.S. Department of Agriculture, Forest Service, Northern Research Station. 161 p.
Tremblay, G.D. and Boudreau, S., 2011. Black spruce regeneration at the treeline ecotone: synergistic impacts of climate change and caribou activity. Canadian Journal of Forest Research, 41(3), pp.460-468.
USGCRP, 2018: Impacts, Risks, and Adaptation in the United States: Fourth National Climate Assessment, Volume II [Reidmiller, D.R., C.W. Avery, D.R. Easterling, K.E. Kunkel, K.L.M. Lewis, T.K. Maycock, and B.C. Stewart (eds.)]. U.S. Global Change Research Program, Washington, DC, USA, 1515 pp.
Violle, C., Navas, M.L., Vile, D., Kazakou, E., Fortunel, C., Hummel, I. and Garnier, E., 2007. Let the concept of trait be functional!. Oikos, 116(5), pp.882-892.
Vose, James M.; Peterson, David L.; Patel-Weynand, Toral, eds. 2012. Effects of climatic variability and change on forest ecosystems: a comprehensive science synthesis for the U.S. forest sector. Gen. Tech. Rep. PNW-GTR-870. Portland, OR: U.S. Department of Agriculture, Forest Service, Pacific Northwest Research Station. 265 p.
Vose, James M.; Clark, James S.; Luce, Charles H.; Patel-Weynand, Toral, eds. 2016. Effects of drought on forests and rangelands in the United States: A comprehensive science synthesis. Gen. Tech. Rep. WO-93b. Washington, DC: U.S. Department of Agriculture, Forest Service, Washington Office. 289 p.
Will, R.E., Wilson, S.M., Zou, C.B. and Hennessey, T.C., 2013. Increased vapor pressure deficit due to higher temperature leads to greater transpiration and faster mortality during drought for tree seedlings common to the forest–grassland ecotone. New Phytologist, 200(2), pp.366-374.
Williams, A.P., Abatzoglou, J.T., Gershunov, A., Guzman‐Morales, J., Bishop, D.A., Balch, J.K. and Lettenmaier, D.P., 2019. Observed impacts of anthropogenic climate change on wildfire in California. Earth’s Future, 7(8), pp.892-910.